Abstract
Three-dimensional (3D) printing is an additive manufacturing process. This technology provides us with the opportunity to create 3D structures by adding material on a layer-by-layer basis, using different kinds of materials such as ceramics, metals, plastics, and polymers. Nowadays, tissue engineering investigations are taking place on a widespread basis in the fields of regeneration, restoration, or replacement of defective or injured functional living organs and tissues. For this reason, it is important to understand the basic concept of 3D bioprinting as a tool for producing a 3D structure combining living cells and biomaterials and controlling cell proliferation, attachment, and migration within 3D structures. There are a variety of applications for additive manufacturing printing technology available to surgeons at this moment, like scaled models for preoperative planning based prosthetics or custom implants and biocompatible scaffolds. Moreover, this technology can be used as a tool to improve surgical and medical education, by using simulation models and utilising its potential to replicate complex anatomy by employing distinct materials that mimic the characteristics of the native tissue in an effort to increase patient safety through repetition of common procedures.
INTRODUCTION
Three-dimensional (3D) printing technology was first introduced in 1986 by Charles W. Hull,1 and named stereolithography.1 Initially, he referred to additive manufacturing and, despite having to start with a slow diffusion, this technology has acquired a major reputation and become widespread over past decades.2-4 The reason for this is the ability to use this technology to design and fabricate complex anatomical structures as a guide for complex anatomical studies, to reconstruct complex organs with intricate 3D microarchitecture and fabricate scaffolds for stem cell differentiation.
3D printing is one of many additive manufacturing processes.5,6 This technology provides us with the opportunity to create 3D structures by adding material layer-by-layer. To do this, different kinds of materials can be used, such as ceramics, metals, plastics, and polymers (synthetic or natural polymers).7 3D modelling software, including computer-aided design (CAD) or computed tomography (CT) images, can help us to design our models, use machine equipment, and layer materials.8 After creating a CAD sketch, 3D printing equipment then reads the data from the CAD file and produces the respective 3D structure.9
There are different types of 3D printing technologies available, such as vat photopolymerisation (hardened with ultraviolet [UV] light), material jetting, material extrusion, powder bed fusion, binder jetting, sheet lamination, and directed energy deposition. The most commonly used technologies within this process are stereolithography apparatus (SLA) and digital light processing.10,11 This method uses a vat of liquid photopolymer resin and produces a 3D structure layer-by-layer cured by a UV laser to create the 3D structures one at a time.12
The most commonly used machine in the material extrusion process is that of fused deposition modelling (FDM).13,14 This is the simplest 3D printing technology and uses a thermoplastic filament as the printing material. The filament is melted in the head of the 3D printer through heating and then 3D structures are created by adding material layer-by-layer. The sheet lamination process includes different materials within the sheet by way of external force. The different materials able to be used to create each sheet include metals, plastics, polymers, etc.15,16 During the sheet lamination process, the sheets are laminated together using heat and pressure and then cut into the desired shape with a laser or blade. Finally, the directed energy deposition process is mostly used in the high-tech metal industries and in rapid manufacturing applications.17,18
CONCEPT OF 3D BIOPRINTING
Nowadays, tissue engineering investigations are widely carried out in the fields of regeneration, restoration, or replacement of defective or injured functional living organs and tissues.19-21 For this reason, it is important to understand the basic concept of 3D bioprinting as a tool to produce a 3D structure combining living cells and biomaterials and controlling cell proliferation, attachment, and migration within 3D structures.
In this way, biomedical scaffolds made of natural or synthetic polymers can be used in biomedical and tissue engineering applications22,23 to replace or regenerate the native tissues functionally and structurally. A scaffold has several functions: it should provide internal pathways for the cell attachment and migration, it must transfer various growth factors and waste products, it should keep its shape while the cells are growing, and have adequate mechanical properties.24 To achieve these functions, biomedical scaffolds for tissue engineering require a highly porous 3D structure that allows cell affinity such as proliferation, migration, attachment, and differentiation, and even enables nutrients and oxygen transport.25,26
Advances introduced by 3D bioprinting have importantly enhanced the ability to control pore size distribution, pore volume, and pore interconnectivity of scaffolds. Furthermore, the development of biomaterials in 3D bioprinting is another important field; presently, different 3D printing processes can mix living cells and bioactive molecules in biomaterials (hydrogels) to make successful 3D structures.
TYPES OF 3D PRINTING METHODS USEFUL IN BIOMEDICAL APPLICATIONS (TABLE 1)
Vat Photopolymerisation Method
The vat photopolymerisation process was patented in 1986 by Charles W. Hull.1 The SLA machine uses UV light to create 3D structures and is based on the vat photopolymerisation principle that monomer resins are photosensitive when exposed to UV light or another similar power source. Photopolymerisation is driven by a chemical reaction that produces free radicals when exposed to certain wavelengths of light. Photons from the light source dissociate the photoinitiator to a high energy radical state and the free radicals induce the polymerisation of the macromer or monomer solution. However, the problem with this photopolymerisation process is that the created free radicals can cause damage to cell membrane, proteins, and nucleic acids. To combat this, hydrogel scaffolds using this technology have been created recently using 3D printing.
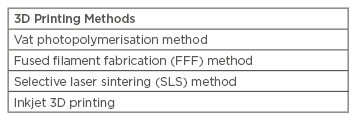
Table 1: Types of 3D printing methods useful in biomedical applications.
Fused Filament Fabrication Method
Fused filament fabrication (FFF) printers use a thermoplastic filament; during the process the filament is heated to its melting point and then extruded to prepare a 3D structure. Thermoplastic filaments are extruded onto the substrate to fabricate a 3D structure. All the procedures are controlled by a computer that translates the dimensions of a structure into X, Y, and Z co-ordinates during printing. This technique is a good and reliable option for fabricating 3D scaffolds in tissue engineering applications and many researchers have reported using this method for tissue engineering. The advantages of this method in tissue engineering applications are: ease of use, the variety of biomaterials, good mechanical properties, and that a solvent is not required. The disadvantages are: material restriction related to thermoplastic polymers and the lack of guarantee that that it can be printed with cells effectively due to the high manufacturing temperature.
Selective Laser Sintering Method
The selective laser sintering (SLS) technique uses a laser as a power source to form solid 3D structures, using a high-powered laser for powder sintering to form a scaffold. This method utilises selective laser printing from 3D modelling software on the surface of a powder bed and may print using several different materials, such as ceramics, metals, and polymers. This technology can be used for tissue engineering, creating different scaffold structures from polymeric biomaterials and their composites, like bone.27 These composite scaffolds are effective at supporting cell adhesion, proliferation, and growth, but have met with limited success in terms of accurately achieving the required porosity levels.28 Other authors have reported a technique to design and manufacture a customised titanium mesh for minimal bone augmentation of an atrophic maxillary arch, guided by the final position of the prosthesis and according to the implants necessary for its support.29 The main advantage of this process for tissue engineering applications is the wide range of biomaterials that can be used. The disadvantage of laser printers is that they tend to be large, cumbersome, and expensive.
Inkjet 3D Printing
Inkjet bioprinters are the most commonly used type of printer for biological and non-biological applications. This method creates different structures using a rapid prototyping and layered manufacturing technology and has seen significant developments in the use of polymeric bioink printing for applications in biological and tissue engineering fields. Different kinds of tissue can be created using printable hydrogels, such as retinal tissue and adipose tissue matrix, among others. The advantages of inkjet 3D bioprinting for tissue engineering applications are: patient-customised fabrication, rapid production, the low cost of production, and ease of incorporating both the drug and biomolecules. In addition, it can be printed with the cells. The main disadvantages are the size limitations, biomaterials available, low resolution, and that it has the worst mechanical properties.
WHERE ARE WE NOW IN THE 3D BIOPRINTING WORLD?
Nowadays, 3D printing technology is rapidly becoming easy and inexpensive enough to be used by doctors, students, and engineers;30,31 the accessibility of downloadable software from online repositories of 3D printing designs has proliferated, largely due to the expanding applications and decreased cost of this technology.31-33 However, processes are limited to scaffolds for cell support and simple body parts such as bone, and currently 3D bioprinting materials are mostly limited to collagen, gelatin, fibrin, ceramics, thermoplastics, or light-curable composites; this is why the most developed applications for 3D bioprinting are used for prosthetic limbs, orthodontic devices, and bone implants.
However, different universities and companies around the world are working hard to develop different lines of research about 3D bioprinting. Organovo (San Diego, California, USA), one of the biggest bioprinting companies, has created liver bioprinted human tissue models with collagen using proprietary 3D bioprinting technology (ExVive™).34 The resulting tissues contain accurate and reproducible 3D structures that can remain completely functional and reliable over 40 days. Other authors report the creation of scaffolds for the human kidney using 3D bioprinting technology,35 while researchers from Cornell University, Ithaca, New York, USA, have reported 3D printed ears similar to the human ear using 3D bioprinting and collagen gels with living cells.36 So far, as mentioned above, patient customised 3D bioprinting has been studied only in a few laboratories.
There are a variety of applications for additive manufacturing printing technologies available to the surgeon. For example: scaled models of the maxillofacial skeleton for preoperative planning based prosthetics (Figure 1) or custom implants, biocompatible scaffolds, an artificial airway for a newborn with tracheobronchomalacia, or the creation of artificial bone using rapid prototyping technology to reconstruct portions of the skull to orbit/midface and mandible, with a variety of uses for presenting pathology including trauma, osteomyelitis, postsurgical deformity, and hemifacial macrosomia, with satisfactory cosmetic outcomes.
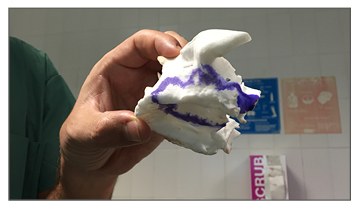
Figure 1: An example of a 3D printed maxillary bone to use as a guide during surgery.
Moreover, some other authors use additive manufacturing 3D printing technology as a tool to improve surgical and medical education, using simulation models and its potential to replicate complex anatomy by employing distinct materials that mimic the characteristics of the native tissue in an effort to increase patient safety through repetition of common procedures.
3D PRINTED ORGANOIDS
Currently, there are several 3D culture methods including scaffold-based models (hydrogels or solid biomaterials) and scaffold-free platforms for spheroid growth. It is likely that 3D culture may provide more reliable cellular models and help to reduce the number of animals used for drug toxicity and efficacy tests.
Newly developed medical treatments of human disease usually have limitations such as individual differences among patients, difficulty with the prediction of outcomes, and time-consuming drug testing. Precision medicine is now coming into focus and becoming more relevant to clinical practice. 3D organoid culture based on a specific disease, and even on a specific individual, is expected to develop into a powerful tool of precision therapy. Primary cancers, infectious diseases, and developmental diseases can be replicated ex vivo on biopsy samples, and these kinds of ‘live’ clinical specimens may become useful for drug testing, gene editing, or for research on prognosis.37
BENEFITS OF 3D BIOPRINTING
Using these devices, physicians have the freedom to produce custom-made prosthetics and implants, and provide positive solutions for patients. Moreover, physicians can have access to more affordable models and increase cost efficiency. Another advantage is the time of production, as only a few hours are needed to develop an implant. Finally, there is the opportunity to democratise technology and expand the possibilities to share concepts about research within this field.
WHAT ARE THE COSTS INVOLVED IN 3D BIOPRINTING?
Customised implants and prosthetics hold significant value for physicians and patients, facilitating improvements in surgical time, surgical tool availability, medical device or surgical success, and patient recovery through the ability to create custom-made devices and surgical tools. These advantages can decrease the length of the patient’s hospital stay, surgical tool costs, and treatment failure costs. This method can help with cost-efficiency owing to its potential for low-cost production of items; however, large-scale production is still cheaper via traditional manufacturing approaches. Recent estimations place savings in the cost of surgery around $100 per minute, as well as reducing the risks of long-term anaesthesia. However, high start-up costs continue to limit the implementation of these strategies.
More recently, USA-based biotech startup Aether (San Francisco, California, USA) and BioBots (Philadelphia, Pennsylvania, USA) released two models of low-cost desktop 3D printers for biomaterials, with each costing around $10,000. These two models use FDM technology to produce bioprinting models and have emerged as an option to spread this type of technology and reduce the cost involved in the production of such models.
LIMITATIONS OF 3D PRINTING FOR BIOMEDICAL APPLICATIONS (TABLE 2)
Expectations surrounding this technology are often exaggerated by the media, governments, and even researchers, who promote unrealistic projections of possibilities; therefore, it is necessary to understand the basic limitations of this technique. Anatomical features and tissue architecture may have details on the scale of hundreds of microns; at the moment it is difficult to achieve this with the standard 3D printers available. Moreover, this problem can limit the ability to create small features that survive the fabrication process, as powder particles must be bound together tightly. Another problem is the limited number of biodegradable, biocompatible resins. Advances have been made to synthesise new macromers with biodegradable moieties; however, these materials have not been US Food and Drug Administration (FDA) approved.

Table 2: Current challenges for cell-tissue printing.
Another problem of 3D printing for biomedical applications are the limited materials available, like collagen, gelatin, fibrin, ceramics, thermoplastics, or light-curable composites. To overcome these limitations, the development of new biomaterials that can be printed in conjunction with cells is necessary. However, these biomaterials should be biocompatible, easily manufactured, and have sufficient mechanical properties for cell support and a secure 3D structure.
WHAT IS THE ACTUAL STATUS OF CELL PRINTING?
The recently announced possibility of bioprinting using stem cells unlocks new possibilities within this domain. The world’s first ever human mesenchymal stem cells bioink is now offered by the Swedish startup company CELLINK® (Gothenberg, Sweden) and the American stem cell company RoosterBio Inc. (Frederick, Maryland, USA).
A report from China has claimed the availability of 3D bioprinting tissue to recreate parts of the kidney, ears, and livers,38 although they do not seem to be implantation-ready. Other reports regarding the bioprinting of bones, cartilage, and muscles as well as other tissues are being conducted.
The 3D bioprinting strategy was initiated by Dr Anthony Attala from Wake Forest Institute for Regenerative Medicine Winston-Salem, North Carolina, USA,39 who applied this technology to manufacture organ tissues for the heart and kidney, and by Gabriel Villard from Oxford University, Oxford, UK, who developed a bioprinter and later, by printing two layers of different cells, for the first time observed changes in specimens after the printing process, later named 4D printing.
Furthermore, one of the major companies in the 3D bioprinting domain is Organovo. Today, it is not yet possible to 3D bioprint any implantable human organ, but at Organovo, they have printed liver and kidney tissues. The printed liver tissue is significantly more effective for drug testing than standard 2D liver culture systems offered by industry as it consists of primary human hepatocytes, stellates, and endothelial cell types, which are found in native human liver enabling drug testing that is stable for at least 42 days. Fully functional printed organs may be possible within the next 10–20 years.38
THE FUTURE OF 3D BIOPRINTING
Nowadays, the 3D printing industry only represents a small proportion of the market; however, in the next 10 years, this proportion is expected to grow. 3D bioprinting has some challenges to overcome, like the development of new biomaterials and improving printers’ technology to advance results.
The current 3D bioprinting challenges for cell/tissue printing are: the design of a bioprinter compatible with physiologically relevant materials and cells, increasing the resolution and speed of these machines and their commercial applications, improving biomaterial composition and cell sources, developing vascular and nerve models, and understanding maturation models for every material. Therefore, it is important to understand the future role robotics and nanomedicine have in the development of this technology also.39 There is a need to improve the biocompatibility and mechanical properties for cell support, which will facilitate the creation of soft tissue and organs that can be directly transplanted into the human body.
The final question surrounds the possibilities of a biofabrication line. A recent analysis published by Mironov et al.40 addressed this question and concluded that the only economic and reasonable way to commercialise organ-printing technology is to systematically employ scalable, automated robotic technology and to build an integrated organ biofabrication line. The biofabrication of a human organ will require the development of a series of integrated automated robotic devices.