Abstract
While the worldwide prevalence of kidney disease is increasing rapidly, the current therapeutic repertoire for these patients is often limited to dialysis and organ transplantation. However, advances in developmental and stem cell biology have highlighted the potential of stem cells for the development of novel renal regeneration therapies. While there are currently no approved stem cell-based treatments for kidney disease, various types of stem cells have been shown to facilitate regeneration of kidney tissue in preclinical models of both acute and chronic kidney injury. This review summarises the current status of stem cell-based therapies to battle kidney disease. In addition, future directions for the clinical translation of stem cell research towards development of novel renal regeneration therapies are discussed.
INTRODUCTION
Organ shortage continues to be the major unresolved problem in transplantation medicine. Although organ transplantation is currently the best treatment to repair organs and tissues that have lost their native function, stem cell-based therapies provide promising alternatives to counteract the shortage of donor organs.1,2 Since the introduction of haematopoietic stem cell transplantation,3 scientific insights into cell-based therapies are gradually finding their way to the clinic and into the patient.4 In recent years, the number of cell-based clinical trials using somatic stem cells or derivatives of pluripotent stem cells (PSC) has markedly increased.4-6 PSC, including embryonic stem cells and induced PSC (iPSC), are expected to be the most promising cell source within the field of regenerative medicine due to their prolonged proliferative potential and multilineage differentiative capacity. Furthermore, human iPSC (hiPSC) can be readily generated from patients, which provide possibilities to develop immunocompatible tissues tailored not only to a specific disease, but also to an individual patient. Recently, several clinical trials have been performed, including those on the topic of transplantation of oligodendrocyte progenitor cells in patients with spinal cord injury,7 transplantation of encapsulated beta cells to battle Type I diabetes mellitus,8 treatment of age-related macular degeneration using retinal pigment epithelium cells,9-11 and transplantation of mesenchymal stem cells (MSC) in patients with graft-versus-host disease (GVHD).12 Additionally, clinical iPSC research is also set to begin for patients with myocardial infarction and Parkinson’s disease in Japan.13
In the field of kidney disease, stem cell-based therapies are the centre of attention for treatment of chronic conditions leading to irreversible kidney failure. Chronic kidney disease (CKD) affects up to 13.4% of the population worldwide and is associated with a serious financial burden, making it a growing public health and economic concern.14 CKD is characterised by gradual, irreversible loss of nephrons, the functional units within the kidney, before eventually progressing towards end-stage renal disease. In turn, patients with end-stage renal disease are dependent on renal replacement therapy, including kidney transplantation or haemodialysis, for survival. However, kidney transplantation has been limited by a shortage of donor kidneys, whereas haemodialysis requires high costs relative to the increase in quality of life and average life expectancy. In this scenario, stem cell-based therapies bear the potential to overcome the above-mentioned limitations, providing not only a cost-effective, but also a long-term treatment option for patients with CKD. For kidney disease, three major strategies for stem cell-based therapies have been proposed (Figure 1). The first strategy takes advantage of the anti-fibrotic, anti-inflammatory, and angiogenic potency of MSC, while the second strategy focusses on the transplantation of kidney-specific progenitor cells differentiated from PSC. The third strategy encompasses the xenotransplantation of animal-derived kidneys. In this review, the current status of these three strategies for stem cell-based therapies to confront kidney failure is summarised. The growing concept of kidney resident stem cells, a pre-existing intratubular stem cell population, also referred to as scattered tubular cells, for kidney regeneration is not included in this review. This cell population has been extensively reviewed elsewhere.15 In addition, the authors discuss the future directions of stem cell-based therapeutics for kidney diseases and alternatives to circumvent the shortage of donor organs in renal transplantation medicine.
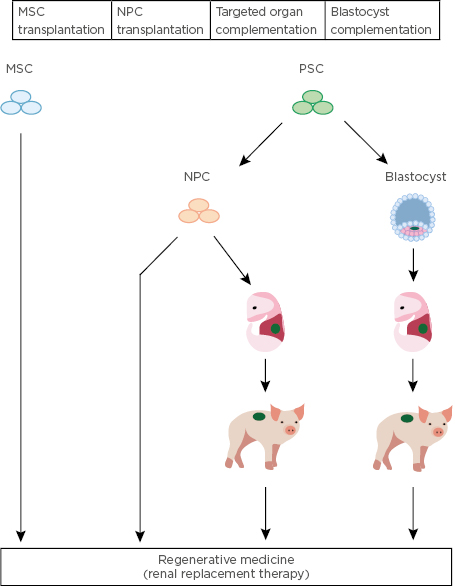
Figure 1: Overview of stem cell-based strategies to treat kidney disease.
Strategies proposed for renal regeneration include the transplantation of mesenchymal stem cells, nephron progenitor cells derived from pluripotent stem cells, and xenotransplantation of animal-derived kidneys following targeted organ or blastocysts complementation. MSC: mesenchymal stem cells; NPC: nephron progenitor cells; PSC: pluripotent stem cells.
MESENCHYMAL STEM CELLS TO TREAT RENAL DISEASE
MSC, a population of rare progenitor cells, were first identified in bone marrow >50 years ago.16,17 MSC are a specialised subset of non-haematopoietic cells, defined by capabilities of self-renewal and differentiation into the mesodermal lineage (giving rise to osteogenic, chondrogenic, and adipogenic cells). Some studies suggested that MSC are also able to differentiate into ectodermal and endodermal lineages,18-20 yet this pluripotent potential of MSC is still under debate.21
Because MSC extracted from bone marrow exhibit heterogeneous characteristics, phenotypic and functional equivalence is required for the use of MSC in any clinical context. The International Society for Cellular Therapy (ISCT) defined three criteria to characterise MSC: 1) MSC must adhere to plastic culture plates under standard tissue culture conditions; 2) MSC should express mesenchymal markers, including CD105, CD73, and CD90, but not haematopoietic markers, such as CD45, CD34, CD14/CD11b, CD79a/CD19, and human leukocyte antigen (HLA)-DR; 3) MSC should have the ability to differentiate into skeletal tissue, osteoblasts, adipocytes, and chondrocytes under in vitro conditions.22
Based on these criteria, MSC have been isolated from a wide variety of fetal and adult tissues, including fetal amniotic fluid, placenta, umbilical cord, adipose tissue, endometrium, bone, kidney, lung, and liver.23 Of note, comparison of gene expression profiles showed considerable differences in MSC phenotype and functional capacity based on their tissue of origin.20,24-26
For therapies using human MSC (hMSC), three distinct mechanisms can be discerned: 1) hMSC differentiate into a variety of cell types, which allow repair or reconstruction of bone tissue and cartilage;21,27-29 2) hMSC can modulate the microenvironment surrounding the injured tissue by secretion of immunosuppressive and anti-inflammatory factors that arrest cell cycle progression of invading immune cells, such as B cells, T cells, and macrophages. These immunomodulatory effects can be used to tackle various immune-related diseases, such as acute GVHD and Crohn’s disease.5,30 Finally, 3) hMSC secrete growth factors, cytokines, and extracellular vesicles that stimulate vascularisation and prevent apoptosis and fibrosis. Based on these secretive functions, hMSC are being explored as a cell-based therapy for myocardial injury, liver cirrhosis, and renovascular disease.31-36
In the field of kidney disease, several preclinical reports have indicated the therapeutic potential of MSC in animal models of acute kidney injury (AKI) and CKD. In these models, bone marrow and adipose-derived MSC showed protective and regenerative effects via paracrine anti-inflammatory, anti-fibrotic, and vascularisation properties.37,38 Accordingly, findings from several studies specified the secretion of extracellular vesicles as a major mechanism of action by which MSC can transfer biological cues to promote regenerative processes in injured renal cells.35-37 In recent years, some clinical studies have investigated the administration of hMSC in patients with AKI and CKD.39 Saad et al.34 assessed the safety of intra-arterial treatment with autologous, adipose-derived hMSC in patients with atherosclerotic renovascular disease. Single infusion of hMSC (1.0×105 or 2.5×105 cells/kg) increased cortical perfusion and renal blood flow, and reduced renal tissue hypoxia, suggesting a potential adjunctive role for hMSC in the management of ischaemic renal disease.34 On the contrary, intra-aortic delivery of hMSC in 156 adult subjects with early-stage AKI who were undergoing cardiac surgery showed no beneficial effects.40 There are several reports that the mechanism of renal repair observed following ischaemia-reperfusion injury does not include replacement of renal tubule cells by injected hMSC.41-44 Instead, cytokines secreted by hMSC exert transient immunosuppressive and regenerative effects, such as increased angiogenesis.34 This would suggest that besides hMSC, other stem cell or progenitor-like cells are necessary to restore kidney tissue. As the regenerative potential of hMSC for kidney disease remains controversial, more clinical evidence is needed to pinpoint the use of hMSC as a treatment for kidney disease.
To establish an effective and stable hMSC-based therapy, several challenges are still looming. The function of hMSC is known to decline with age, as the culture expansion needed before hMSC transplantation is associated with cellular senescence. Therefore, recommendations for the clinical use of hMSC are restricted to 3–5 passages.45,46 In a Phase II clinical trial, Le Blanc et al. showed that infusion of early-passage hMSC into patients with GVHD resulted in a better outcome.30 Additionally, clonal expansion of hMSC in culture is not efficient to produce sufficient numbers of hMSC needed for transplantation. As such, nonclonal expansion of a heterogeneous population of hMSC is more likely to be adopted in a clinical setting, despite variations in efficacy.
RENAL REGENERATION USING PLURIPOTENT STEM CELLS
PSC are at the forefront of regenerative medicine in kidney disease by virtue of their unlimited self-renewal and capacity to differentiate into all types of renal cells, which provides possibilities to overcome the current shortage of donor kidneys. The kidney consists of various types of cells, but its major progenitors are classified into three groups: nephron progenitor cells (NPC; SIX2+),47 ureteric buds (GATA3+),48 and interstitial stromal progenitor cells (FOXD1+).49 Regarding kidney regeneration, Harari-Steinberg et al.50 showed that engrafted human fetal NPC improve kidney function in a murine 5/6 nephrectomy kidney injury model.50 However, as the use of human fetus-derived NPC brings along ethical questions, PSC derived from reprogrammed somatic cells are likely to avoid any ethical issues.
There are a few reports that demonstrate the therapeutic effect of hiPSC-derived NPC. Toyohara et al.51 reported that renal subcapsular transplantation of hiPSC-derived OSR1+SIX2+ renal progenitor cells (RPC) improves blood urea nitrogen and serum creatinine levels and ameliorates histopathological changes in a murine AKI model induced by ischaemia/reperfusion injury, whereas parenchymal injection of RPC did not show any therapeutic effects despite the cell engraftment.51 As there was no evidence of integration of transplanted cells into the mouse kidneys, the observed therapeutic effect of renal subcapsular transplantation of RPC was anticipated to be due to renoprotective factors secreted by RPC, such as angiopoietin-1, vascular endothelial growth factor (VEGF), and hepatocyte growth factor. On the contrary, Imberti et al.52 reported that hiPSC-derived RPC administrated via tail vein injection could engraft into damaged kidneys and restore renal function in mice with cisplatin-induced AKI.52 Differences in engraftment efficiency between these studies might be influenced by the mechanism of AKI-induction, the route of administration of the RPC, and the quality of the transplanted RPC (depending on the differentiation induction method). The results of these studies suggest that the transplantation site plays a crucial role in the therapeutic effect, rather than progenitor cell engraftment. However, renal regeneration by secreted renoprotective factors relies on the kidney’s intrinsic potential for structural repair or true regeneration, which is limited for the human kidney. To functionally restore or regenerate nephron structures within injured kidneys, engraftment of progenitor-like cells is indispensable.50
In the past decade, in-depth knowledge of mammalian kidney development has been translated into significant advances regarding directed differentiation of hiPSC into cells of the kidney lineage.48.53-57 NPC derived from PSC possess the developmental potential of their in vivo counterparts and form renal vesicles under three-dimensional (3D) culture conditions, eventually self-organising into nephron structures. These so-called kidney organoids closely mimic the organisation of kidney epithelia, including structures expressing markers of podocytes, proximal tubules, loops of Henle, and distal tubules. Kidney organoids have been shown to facilitate the interrogation of renal toxicity, disease modelling, and mechanistic studies into human kidney development.54,58-64 As such, it is hypothesised that nephron structures differentiated from these NPC might function in vivo as well. In fact, NPC have been reported to form functional glomeruli and tubular structures in vivo.65-68 An overview of all studies exploring transplantation of NPC or NPC-derived tissues is provided in Table 1.
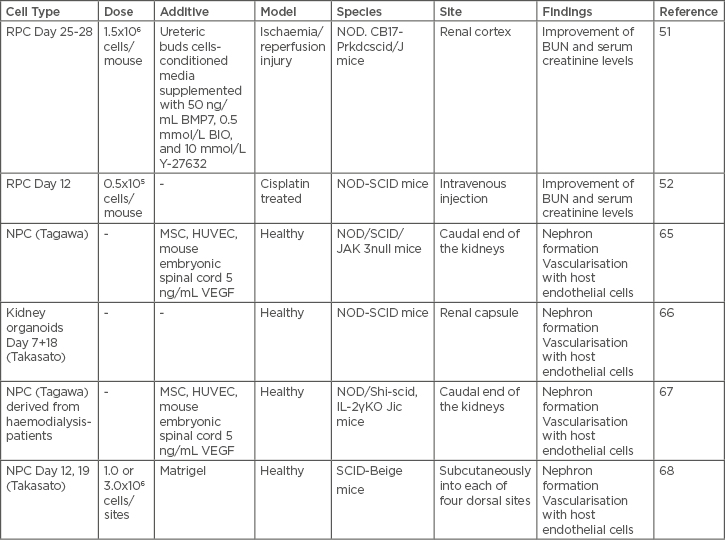
Table 1: Overview of studies exploring in vivo transplantation of renal progenitor cell, nephron progenitor cell, or nephron progenitor cell-derived tissues.
BMP7: bone morphogenetic protein 7; BUN: blood urea nitrogen; HUVEC: human umbilical vein endothelial cells; MSC: mesenchymal stem cell; NOD: nonobese diabetic; NPC: nephron progenitor cell; RPC: renal progenitor cell; SCID: severe combined immunodeficiency; VEGF: vascular endothelial growth factor.
The study conducted by Sharmin et al.65 described a novel transplantation method in which spacers were used to release the tension of host kidney capsules, which resulted in the differentiation of hiPSC-derived NPC into glomeruli.65 Here, iPSC-derived nephron aggregates were cultured with mouse embryonic spinal cords to initiate tubulogenesis, after which cotransplantation of mixed aggregates of human umbilical vein endothelial cells and MSC allowed the formed glomeruli to connect with the blood vessels of the host and develop podocyte-specific features such as primary processes coupled with slit diaphragm-like structures. This suggests that not only targeted stem or progenitor cells, but also additional cell types need to be included to optimise efficient transplantation. On the other hand, Van den Berg et al.66 succeeded in the formation of functional glomeruli and highly polarised renal tubules upon renal subcapsular transplantation of PSC-derived kidney organoids in mice.66 Mouse endothelial cells were observed in glomerular structures within the transplanted organoids, indicating the construction of vascular networks. While both groups tried to enhance vascularisation by addition of VEGF, no additional effect of exogenous VEGF was observed. Together with gene expression data showing VEGF expression in podocytes, this might suggest that transplanted podocytes sufficiently provide endogenous attractants to support vasculogenesis in and around engrafted kidney tissues.
Another important factor for efficient regeneration using NPC concerns the timing of transplantation. Whereas Sharmin et al.65 transplanted NPC 1 day after spinal cord induction, Van den Berg et al.66 transplanted kidney organoids formed by NPC after 18 days of CHIR99021 treatment. Furthermore, Bantounas et al.68 reported that NPC differentiated for 12 days developed better nephron-like structures compared to NPC differentiated for 19 days when transplanted subcutaneously in mice.68 Transplantation of NPC on Day 12 after induction of differentiation resulted in substantially more mature nephron structures compared to the structures formed in 3D organoids cultured in vitro for 12 weeks. Although no teratoma-like structures were observed in the clusters of engrafted NPC, grafts also contained cartilage and poorly differentiated kidney tissue. As the presence of heterologous cells may cause uncontrolled tissue formation, eventually leading to side effects during treatment, strict quality control of NPC prior to transplantation is of the utmost importance. Accordingly, a recent study of Phipson et al.69 employed single-cell RNA sequencing to display the batch-to-batch variation in kidney organoid generation.69 This underscores the importance of quality control measures and stable differentiation protocols before adopting kidney organoids for use in regenerative medicine. Recently, the Morizane group has developed a chemically defined protocol for differentiation of hiPSC into SIX2+ NPC with 90% efficiency. These NPC further possess the developmental potential to form organoids containing epithelial nephron-like structures expressing markers of podocytes, proximal tubules, loops of Henle, and distal tubules in an organised, continuous arrangement that resembles the nephron in vivo.54,70 In this differentiation protocol, SIX2 immunostaining could be used as an intermediate quality control measure during organoid generation. However, depending on the protocol, more markers that could evaluate the differentiation status of hiPSC or induced NPC need to be established.
Although it has been argued that renal cells derived from PSC are immature, mostly reflecting first or second trimester equivalents, and therefore lack functional aspects of the native tissue, novel bioengineering methods have been explored to obtain more mature and functionally active kidney tissue.71-73 The Lewis group reported an elegant method that combines bioprinting, 3D cell culture, and microfluidics to recreate the geometry and architectural complexity of kidney tubules.71 The perfusable tubules within this model not only contained characteristic structural features, such as 3D convolution and open luminal architecture, but also expressed a variety of transporter proteins. More recently, the Morizane group adapted this method, in collaboration with the Lewis group, to enhance vascularisation and maturation of kidney organoids by applying fluidic shear stress during the later stages of the differentiation protocol.74 The ability to induce vascularisation and morphological maturation of kidney organoids opens new avenues for therapies for renal regeneration.
However, a remaining challenge within renal regeneration comprises the formation of collecting ducts and connecting structures for drainage of urine. Taguchi et al.56 developed a method to induce the ureteric bud from hiPSC, the precursor to the adult kidney collecting system which arises from the anterior intermediate mesoderm. Further co-culture experiments of ureteric bud and NPC in Matrigel (Corning Life Sciences and BD Biosciences) showed branching morphogenesis to some extent.75 Despite the enormous potential of PSC-derived kidney cells and tissues for renal regeneration, further studies are required to optimise the conditions (e.g., timing, cell state, transplantation site) for efficient transplantation (Figure 2).
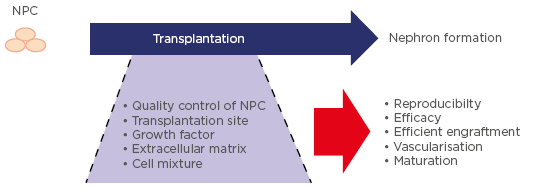
Figure 2: Future directions for optimisation of nephron progenitor cell-based transplantation to regenerate nephrogenic tissue.
NPC: nephron progenitor cell.
CROSS-SPECIES ORGAN GENERATION AND XENOTRANSPLANTATION
Although research on kidney organoids using PSC has dramatically advanced, the challenge of generating whole functional organs for transplantation remains. Blastocyst complementation is one such method in which a desired organ of human origin can be generated by integration of human PSC in animal host embryos. In 1993, Chen et al.76 restored lymphocyte development in Rag2-deficient mice (missing the inability to initiate V(D)J recombination needed for immunoglobin rearrangement) by injection of normal embryonic stem cells into the blastocysts of these mice.76 The Nakauchi group created insulin-secreting rat PSC-derived pancreata in Pdx1-/- (pancreatogenesis-disabled) mice.77
Furthermore, the same group generated mouse PSC-derived pancreata in rats that could improve streptozotocin-induced diabetes by transplantation of the mouse–rat chimeric pancreata into mouse kidney subcapsules.78 Transplanted pancreata normalised and controlled host blood glucose levels for >1 year in the absence of immunosuppressants (excluding 5 days of tacrolimus treatment after transplantation). Later, the Nakauchi group showed that the same approach can also be used in larger animals by generating exogenic pancreata from porcine PSC in apancreatic cloned pigs.79 Recently, Wu et al.80 reported that some types of hiPSC exhibit chimeric competency when introduced in post-implantation pig embryos.80 Altogether, these studies reflect the therapeutic potential of interspecies blastocysts complementation for organ regeneration.
For the kidney, the same approach was extended to generate PSC-derived kidneys using Sall1-/- (nephrogenesis-disabled) mouse blastocysts.81 However, nephrogenic tissues not under the influence of Sall1 expression, such as collecting ducts and microvasculature, were not complemented by the injected mouse PSC. Furthermore, whereas the authors were unable to obtain rat PSC-derived kidney in anephric Sall1-/- mice, conversely, mouse PSC-derived kidney tissue could be formed in the metanephric mesenchyme of Sall1 mutant rats.82 Here, the newly-formed kidney tissue showed the expression of several key functional markers and proper ureter-bladder connections were formed, indicating the ability for urine excretion. Unfortunately, postnatal lethality of both Sall1-/-rats and mice complemented with mouse PSC-derived kidney tissue hinders any functional examination of the PSC-derived kidney tissue. As Sall1 expression is not limited to the kidney, but also found in brain, lung, and endocrine tissues, knockout of Sall1 might result in early-onset lethality. As a next step, disruption of alternative genes essential for kidney development should be examined.
Although blastocyst complementation holds great promise for regenerative medicine, the generation of human-animal chimeras might raise important ethical questions. This specifically applies to the use of human cells related to neural and germ lineages. Alternatively, methods ensuring that injected stem or progenitor-like cells solely develop as the desired target organ have been proposed. In these targeted organ complementation methods, precursor cells are transplanted into embryos lacking the specific target tissue. Using this method, Yamanaka et al.83 specifically removed Six2+ NPC from mouse embryos followed by transplantation with rat NPC, resulting in the development of mature kidney tissue, including blood flow and connection with the mouse ureter.83 Using the blastocyst complementation method with PSC, xenotransplantation of rat kidney tissue to mice could not be successfully established. This might suggest that the use of more differentiated progenitor cells (e.g., SIX2+ NPC) improves interspecies kidney complementation. While rodent chimaeras provide a platform for detailed investigation of factors that impact efficient xenotransplantation, larger host animals will be required to generate appropriately sized humanised organs. As sheep and pigs are considered as potential hosts for the development of humanised kidneys, major challenges remain regarding the evolutionary divergence between human donor cells and the host embryonic environment. As such, species differences in the embryologic development of the kidney and the corresponding nephrogenic niche first need to be clarified to facilitate efficient strategies for the generation of humanised kidneys.
CONCLUSION
This review described the progress of stem cell-based technologies with an emphasis on their potential for the regeneration of kidney tissue. Although hMSC are advisable as a cell source for stem-cell based therapies in terms of safety, adequate kidney regeneration is difficult upon hMSC treatment. Therefore, strategies using other stem cell sources need to be developed. The diversity of functions that the kidney exerts in the human body relies on higher-order structures, including advanced tubular segments, microvasculature and a collecting system. In recent years, developmental studies have provided valuable insights in different stages of nephrogenesis along with the involved progenitor cells. Accordingly, several methods for differentiation of NPC and kidney organoids from PSC have been established, which thus far have enabled the formation of vascularised nephrons in vivo. Adoption of bioengineering platforms has shown to facilitate evaluation of NPC and kidney organoids in a controllable in vitro setting, allowing further clarification of the underlying developmental processes required for regeneration of functional kidney tissue. However, to produce human-sized kidney grafts, scale-up of stem-cell derived tissue might involve blastocysts or targeted organ complementation methods in larger animals. While most of these studies are currently performed in smaller animals, such as rodents, progress has been made at an astonishing rate. Altogether, these concerted efforts are opening the door towards a new generation of therapies for renal regeneration.