Abstract
An increasing number of cancer patients have found new hope in the form of recently discovered cell immunotherapies. These novel treatments empower our own immune system to fight and eradicate the malignant tumours, even in metastatic stages. Unprecedented outcomes have been demonstrated with bioengineered cells especially modified in laboratories to trigger a highly specific and active anti-tumour function; however, a main bottleneck for the implementation of these promising therapies resides in the old-fashioned and limited techniques employed for the in vitroanalysis and study of cells during manufacturing and testing processes. Optical label-free biosensors are currently arising as promising analytical technologies for a simplified and accurate cell analysis. In particular, biosensors based on evanescent field principles enable direct, non-invasive, and real-time monitoring of biological events, showing outstanding sensitivities and excellent robustness. Furthermore, these biosensors are easily incorporated in lab-on-a-chip platforms for simple operation and implementation in clinical laboratories. In this review, the authors present and discuss the more recent research aimed to develop label-free optical biosensors for the analysis of live cells and the direct benefits offered to cell immunotherapy production allowing for its widespread implementation.
THE REVOLUTION OF CANCER IMMUNOTHERAPIES
Cancer immunotherapy has been profiled as one of the major breakthroughs of the decade and will probably remain a paramount biomedical landmark in decades to come. While it has long been a pursued goal in immunology and oncology, only now are the first clinical trials showing significant and impressive results in the treatment of cancer patients.1This relatively new therapy seeks the activation, inhibition, or improvement of the immune system to fight cancer. Instead of directly targeting tumour cells, such as conventional radiation therapy or chemotherapy, immunotherapy approaches empower the patient’s natural immune system to attack them. Therefore, the benefits promised by cancer immunotherapies include not only a remarkable increase of treatment efficiencies, but also fewer side effects, and the possibility to treat certain cancer types that do not respond to other therapies or are in advanced stages.
Immunotherapy strategies can be categorised into three broad groups: passive immunomodulation, active immunisation (i.e., cancer vaccines), and the adoptive cell transfer approaches. The first passive immunomodulatory treatments were approved by the U.S. Food and Drug Administration (FDA) in the 1990s and are widely available for cancer patients. This type of immunotherapy consists of the administration of cytokines and other molecules that boost the general immune response of the body to attack the malignant cells. Among them, the administration of IL-2, a T cell growth factor, is used to stimulate endogenous tumour-reactive cells in vivoand has shown reproducible regression of solid human cancers. More recently, a new immunomodulatory approach has emerged, which makes use of monoclonal antibodies to inhibit the immune checkpoints that prevent T cells to identify and eliminate the tumour cells.2The blockade of specific checkpoint molecules, such as cytotoxic T-lymphocyte-associated 4 (CTLA4) or programmed-death 1 (PD1), have shown impressive results for the treatment of different malignancies, such as bladder cancer, non-small cell lung cancer, and cutaneous melanoma. In fact, the innovation of these approaches was awarded with the Nobel Prize in Medicine in 2018. However, because these drugs interfere with the patient immune system in a non-specific manner, the treatment is far from reaching optimum efficacy and probably causes severe side effects.
Instead, a more active immunotherapy could provide better outcomes. Therapeutic cancer vaccines are generally intended to treat growing tumours by strengthening the immune response of the patient to selectively attack the malignant cells.3For that purpose, the vaccines are designed to activate the T cells by presenting specific tumour-associated antigens, which are proteins or peptides solely or over-expressed in cancer cells. Several vaccination strategies are currently under development or being evaluated in clinical and preclinical trials, including protein and peptide vaccines, genetic vaccines (DNA, RNA, and viral), and cell vaccines (tumour or immune cells). This therapeutic immunisation has demonstrated minimal toxicity in all clinical studies and also offers a long-lasting protection against tumour appearance and progression. The main challenge to cancer vaccines relies on the immunosuppressive tumour microenvironment that prevents the immune cells to act and proliferate and, therefore, suggests that vaccines should be administered in combination with other agents or approaches to synergistically engage the anti-tumour response.
Finally, one of the most attractive and encouraging immunotherapies is the so-called adoptive cell therapy.4This strategy consists of identifying and/or engineering the patient’s own T lymphocytes to enhance the anti-tumour activity, expand them ex vivo, and reinfuse them again into the patient with cancer (Figure 1). The massive transfer of these powerful cells into the patient’s body has demonstrated an almost immediate tumour regression and eradication while offering a long-lasting protection against cancer recurrence. Furthermore, because the treatment employs the patient’s own cells, the associated side effects and toxicity could be minimal.
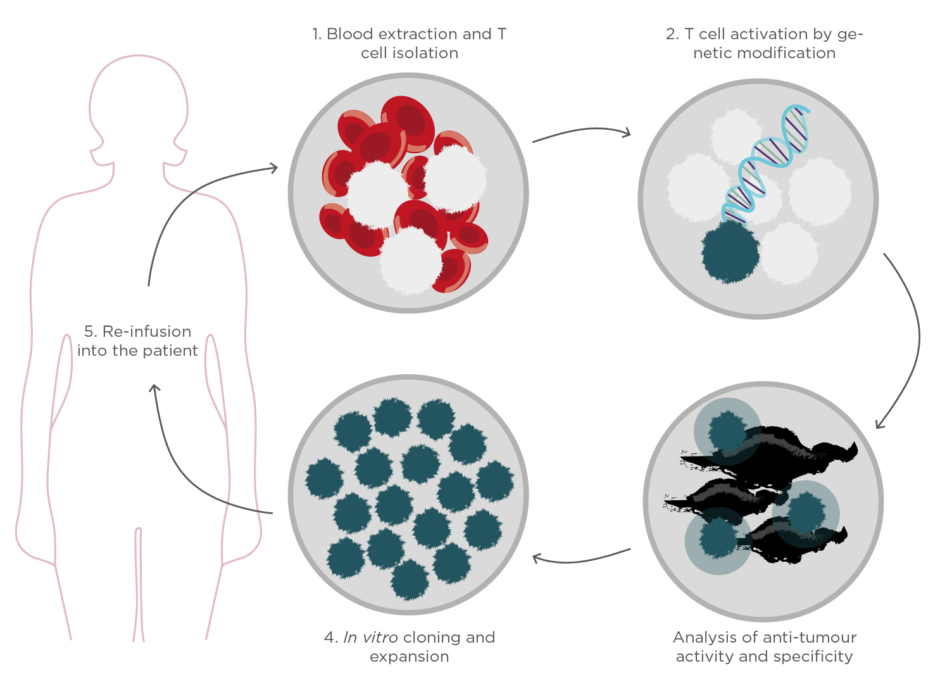
Figure 1. Schematic representation of the production process of cell immunotherapies based on adoptive
cell transfer.
This article briefly reviews the current biomedical challenges associated with cell immunotherapies, especially during the production and evaluation processes, and how they can limit their widespread administration to cancer patients. The authors also introduce optical biosensor nanotechnologies as pioneering and powerful platforms to boost and facilitate the manufacturing and implementation of such personalised medicine, enabling a highly accurate, faster, and simpler analysis of live cells and immune system activity.
CELL IMMUNOTHERAPIES: PROMISES AND CHALLENGES
There are two main types of cell-based immunotherapies depending on whether they use the patient’s own tumour infiltrating lymphocytes (TIL) or artificially bioengineered T cells carrying a chimeric antigen receptor (CAR) on the cell surface.
Autologous TIL are immune cells that have recognised and infiltrated the tumour to eradicate it but have been inactivated by the effect of the tumour microenvironment and cancer immunosuppressive strategies. These lymphocytes can be extracted from the tumour peripheral blood, expanded ex vivo, and stimulated to reinforce their anti-tumour immune activity.5Prior to reinfusion, the patient undergoes a previous lymphodepletion procedure to eliminate endogenous lymphocytes that can compete with activated TIL. Additionally, the cell transfer is usually aided by co-adjuvants, such as IL-2, to enhance the anti-tumour performance. The adoptive TIL therapy has so far demonstrated outstanding response rates in melanoma, even in metastatic stages. Ongoing trials for breast, ovarian, or colorectal cancer are showing promising results when combined with costimulatory ligands or other immunotherapeutic strategies; however, the broad applicability of TIL therapy is limited because not all tumours yield to immunologically active T cells and not all cancer patients have TIL. Identifying antigen-specific T cells with effective anti-tumour function in blood samples is an arduous task in clinical and biomedical laboratories. Another important limitation relates to the manufacturing process of the therapy. TIL are usually grown with multiple rounds of expansion using cytokines or other stimuli, and it has been shown that their immune activity and anti-tumour efficiency is seriously reduced after the long-term ex vivo cultures. Reducing the number of expansion cycles during therapy production is a key factor to enhance the final effectivity and performance of the immune treatment.
An alternative strategy is providing the most exciting results for cell immunotherapies. In the so-called ‘CAR-T’ cell therapy, T lymphocytes from the patients are modified with artificial surface receptors (i.e., CAR) that consist of an antibody-derived ligand fused with the T cell receptor signalling machinery.6A CAR moiety therefore combines a specific antigen binding domain that confers recognition of a tumour-specific antigen, with intracellular signalling motifs that promote T cell activation. The main advantage of CAR-T cell therapy in comparison with the TIL strategy is that the CAR-T cells can be bioengineered and produced from a small number of T lymphocytes, independently of their native anti-tumour avidity. Two of these immunotherapies have been already approved by the FDA for treating blood cancers (i.e., acute lymphoblastic leukaemia and aggressive B-cell non-Hodgkin lymphoma). The European Medicines Agency (EMA) has also started the approval procedure for these CAR-T cell therapies. In parallel, many laboratories worldwide are intensively working on developing, optimising, and evaluating new CAR-T cell therapies for attacking solid tumours; however, relevant success has not yet been accomplished, probably due to the multiple immunosuppressive mechanisms that T cells find within the tumour microenvironment. As well as this, CAR-T cell therapy has been associated with cytokine release syndrome in patients. The rapid activation and expansion of these T cells upon infusion may trigger an immune system reaction that can yield to fever, organ dysfunction, neurologic symptoms, and other toxicities. To manage these serious side effects, the use of corticosteroids or cytokine receptor blockers along with the immunotherapy is being tested, with promising results so far.
Beyond the particular biomedical challenges, cell immunotherapies need to face important obstacles that hamper their widespread implementation and administration.7,8The manufacturing and evaluation process of cell immunotherapies involves multiple and highly specialised procedures, including labour-intensive cultures and routine performance of cell analyses to verify immune responses and anti-tumour activity before re-infusion into the patient(Figure 1). Currently, the analysis of the live cell functionality in clinical and research laboratories is based on fluorescent or colorimetric techniques, such as flow cytometry and enzyme-linked immunosorbent assays (ELISA). These methodologies have demonstrated outstanding sensitivities for analysing and sorting cell phenotypes according to protein expression and secretion; however, they experience important constraints in terms of automation, time resolution, cost efficiency, and cell viability. The need for fluorescent and colorimetric labels for the detection not only compromises cell activity and recovery after the study, but also limits the analysis to endpoint results and impedes the integration in appropriate culture environments. Techniques such as flow cytometry require initial large amounts of cells, which usually imply previous in vitroexpansion procedures that reduce the eventual anti-tumour efficiency of the cells and inevitably delay the therapy administration to the patient.
Herein, the introduction of optical biosensor nanotechnologies to the field of cell analysis could bring an opportunity for accelerating the production process of cell immunotherapies, improving the evaluation accuracies, and also reducing the final costs of the treatment.
OPTICAL BIOSENSORS FOR LABEL-FREE AND REAL-TIME ANALYSIS
Optical biosensors exploit the electromagnetic properties of light for the detection, quantification, and analysis of biochemical interactions with a high sensitivity, and with capabilities for label-free and real-time format assays.9,10Optical label-free biosensors are generally based on the evanescent wave techniques, which enables the detection of minute refractive index variations caused by changes of mass within an evanescent electromagnetic field that is generated at the interface between the sensor and the sample (100–500 nm). The two most common configurations are the surface plasmon resonance (SPR)-based biosensors and the waveguide-based technologies, such as resonators or interferometers (Figure 2). SPR systems employ a nanometre thin layer of gold (45–50 nm) as sensor surface. Upon illumination at certain angle and wavelength, the free electrons of the metal start oscillating collectively creating the so-called plasmon resonance, which in turn generates an evanescent wave that penetrates in the dielectric medium and propagates a small distance along the surface.
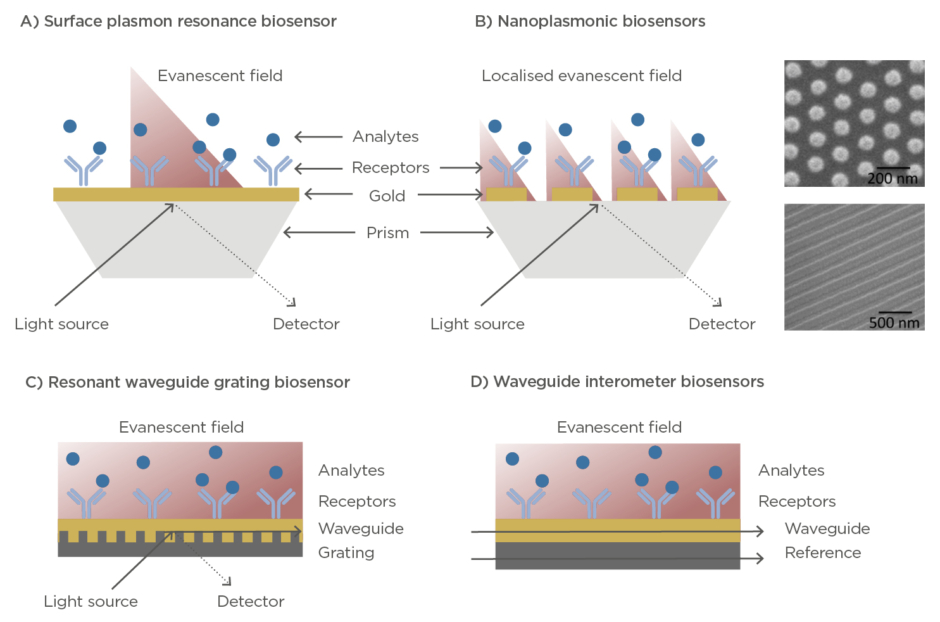
Figure 2: (A) Illustration of a surface plasmon resonance biosensor working in prism-coupling configuration. (B) Illustration of a localised surface plasmon resonance biosensor working in prism-coupling configuration. Right panels show scanning electron microscopy images of different nanoplasmonic structures. (C) Illustration of a resonant waveguide grating biosensor in angular configuration. (D) Illustration of a waveguide
interferometer biosensor.
The plasmon resonance is characterised by the appearance of an absorption dip in the reflected light. By measuring variations in the angle, wavelength, or intensity of the SPR dip, it is possible to monitor in real time biological events occurring on the sensor surface.11SPR biosensors are widely commercially available and are employed in many research laboratories and pharmaceutical industries to carry out routine analysis of biomolecular interactions, kinetic analysis, or drug discovery and evaluation assays.11–14Accompanied by the progress in nanotechnology in the last decade, more sophisticated versions of plasmonic biosensors have appeared and exponentially arose. Nanoplasmonic biosensors incorporate nanostructured surfaces such as nanodiscs, nanorods, nanoslits, and nanoholes that exhibit a localised surface plasmon resonance and provide exceptional features that can be translated in enhanced sensitivities, multiplexing capabilities, or higher miniaturisation and integration adaptability.10,15,16On the other hand, waveguide-based technologies are based on the leaky electromagnetic mode of light travelling along a waveguide material. The resonant waveguide grating (RWG) biosensors are commonly used and commercially available. In this platform, the light is coupled to a waveguide via grating structures, where it propagates through generating an evanescent field and resulting in a narrowband of reflected or transmitted wavelengths detected at the output.17RWG biosensors have been successfully integrated in multiwell plates and imaging configuration for high-throughput and multiplexed screening.18In parallel to RWG systems, waveguide-based interferometers have also been realised, achieving outstanding sensitivities for label-free detection. Here, the measuring guided light mode, in contact with the sample, is combined to a reference light creating an interference. The differences in phase are used to detect and quantify biochemical interactions occurring at the sensor area.19Some examples of waveguide-based sensors are the well-known Mach-Zehnder interferometers,20the bimodal waveguide interferometers,21or the grating-coupled interferometers.22
Over the last years, there has been a steady increase in the number of scientific publications and patents in the area of optical label-free biosensors for the detection of numerous molecules or pathogens, such as bacteria, viruses, DNA or RNA, proteins, peptides, or small molecules like drugs or neurotransmitters.10,11,19,23Surprisingly, the application of optical label-free platforms for live cell biology remains relatively unexplored.24,25The predominate challenges are related to the lack of an appropriate biochemical design for providing clinically relevant information;the use of conventional microfluidic systems being unsuitable for cell culture and long-term analysis; and the inherent limitations of mass-related sensing of optical label-free methods, which commonly yields to insufficient sensitivity for small biomolecule detection and selectivity issues when evaluating complex fluids, such as culture media or blood serum and plasma. Nevertheless, a few studies have investigated the use of label-free optical sensing technology for the analysis of cell functionality in real time, showing promising potential, especially for the implementation in cell immunotherapy development.
OPTICAL LABEL-FREE BIOSENSORS FOR CELL IMMUNOTHERAPY APPLICATIONS
Two critical steps in the cell immunotherapy development process could be greatly improved with the introduction of optical sensing technologies: the detection and identification of relevant immune cells from human samples, and the evaluation of the immune cell activity and tumour response. The high sensitivity, label-free format, and real-time analysis capabilities of optical biosensors might enable the rapid isolation of specific T lymphocytes with anti-tumour reactivity directly from tumour peripheral blood samples, without the need for previous expansion steps and avoiding cell staining with fluorescent markers. On the other hand, retrieving real-time and quantitative information of cell activity events, such as membrane receptor activity or protein secretion, provides direct insights in cell status and functionality.
For example, cancer cells secrete cytokines to the tumour microenvironment in order to grow and proliferate, or to inhibit the action of immune cells trying to attack them. Likewise, immune T cells can release different types of proteins (e.g., cytokines, interferons, necrosis factors, and enzymes) for either killing tumour cells or as a signalling tool for recruiting other immune cells and enhancing the immune response. Thus, the label-free and real-time analysis of cell secretion can facilitate the evaluation of immune cell activation upon interaction with tumour antigens, the cancer cell response, or the study of immunosuppressive strategies in tumour microenvironments (Figure 3A).
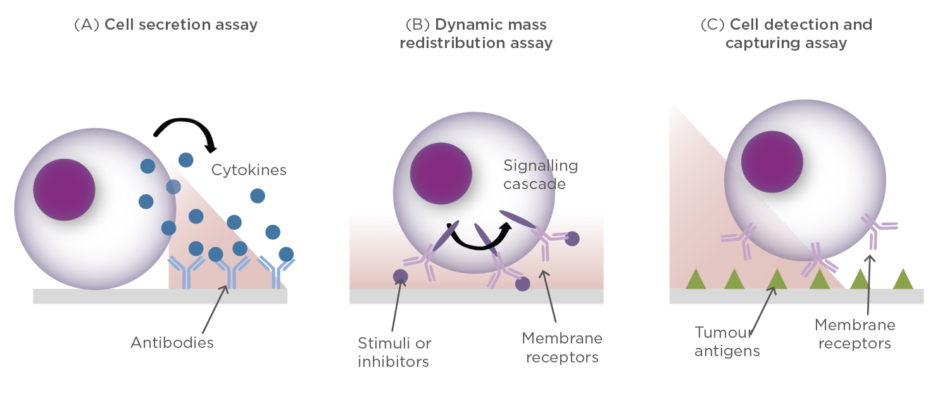
Figure 3: Conceptual illustrations of different cell assays performed with optical label-free biosensors.
(A) Detection of protein secretion; (B) Detection of cytosolic dynamic mass redistribution (DMR); (C) Detection and capture of specific anti-tumour cells.
For the study of cancer cell activity and tumour progression, Liu et al.26developed a SPR biosensor able to monitor, in real time, the secretion of vascular endothelial growth factor (VEGF), a cytokine responsible for promoting the formation of blood vessels and tumour spread. The SPR system incorporated a mini cell culture module within the microfluidics that was placed close to the sensor surface. Ovarian carcinoma cells were stimulated with a calcium ionophore and the secretion of VEGF was readily detected by specific antibodies immobilised on the sensor surface. Li et al.27developed a more advanced lab-on-a-chip nanoplasmonic system for VEGF secretion analysis from live HeLa cells. The platform consisted of two interconnected modules: a microfluidic cell module, where cells could be cultured with appropriate temperature and humidity conditions, and the optical sensor module based on gold nanohole arrays. The nanoplasmonic sensor with multiplexing adaptability monitored the culture cell supernatant for up to 10 hours and was able to detect VEGF secretion from cancer cells at approximately 6 hours after stimulation.
For the analysis of immune cell activity, some promising approaches have also been proposed. Oh et al.28demonstrated for the first time the detection of cytokine secretion from immune T cells directly isolated from human blood. Their system was based on a nanoplasmonic surface surrounded by a microfluidic cell chamber only separated by a micropillar array. After cell incubation and stimulation, they could monitor the detection of TNF-α directly secreted by CD45 cells. With this platform, they could perform a cellular function immunoanalysis with minimal blood sample volume (3 µL) and a total assay time three times shorter than conventional ELISA. Later, the same group demonstrated the multiplexed analysis of different cytokines (IL-2, IFN-γ, and TNF-α) employing a gold nanorod-based biosensor.29Although in this system the cells were not cultured in situ, they proved the potential of nanoplasmonic biosensors for immune cell profiling in a rapid and accurate mode. Pursuing the sensitivity limits of nanoplasmonics, researchers have also attempted single-cell secretion analysis. Raphael et al.30developed a gold nanodisc biosensor and showed real-time detection of antibody secretion directly from an individual hybridoma cell. More recently, Li et al.31employed a gold nanohole array sensor integrated with a valve-gated microfluidic chamber to monitor IL-2 secretion from isolated T cells. The optofluidic system enabled not only the ultrasensitive detection of the cytokine secretion, but also the spatio-temporal profiling of the secretion event, revealing large heterogeneity and behaviour differences between various cells from the same cell line.
Another approach for elucidating cell activity is by measuring the triggering of membrane receptor signalling. When live cells are placed on the sensor surface, the evanescent field is able to probe changes of mass distribution inside the cytosol of the cell (i.e., dynamic mass redistribution), such as those occurring when the signalling is activated (Figure 3B). This strategy, however, requires the evanescent field to propagate over large areas ensuring maximum coverage of the cell entity; therefore, waveguide-based biosensors are better suited for this application. Fang et al.32,33employed a RWG biosensor with angular modulation to monitor the EGF-receptor activation in cancer cells. Upon stimulation with EGF molecules, they were able to detect mass redistribution inside living cells in real time, which was attributed to the molecular rearrangements in the cell during the signalling cascade; however, because EFG stimulation is known to lead to cell rounding and detachment, it is also conceivable that the biosensor was monitoring cell detachment and re-adhesion to the surface. The same approach was employed by Kurucz et al.34to monitor the response of human B cells upon stimulation and inhibition of immune receptors. In this case, non-adherent B cells were immobilised on the surface via electrostatic interactions and the dynamic mass redistribution was measured when cells were stimulated with B-cell receptor antibodies, as well as stimulation inhibitors.
Finally, compared to cell activity analysis, capturing and isolating specific immune cells from complex samples is far more challenging in optical label-free biosensors. In order to selectively capture tumour-reactive immune cells, it is necessary to employ specific tumour receptors as biorecognition elements. Unfortunately, the binding strength between T cells and tumour receptors is rather weak, compared to, for instance, antibody-antigen interactions (Figure 3C). In this regard, Soler et al.35developed a biomimetic SPR biosensor in a recent study for the detection of T cells through interaction with tumour-specific receptors (Figure 3). By monitoring the interaction in real time, they were able to extract kinetic and affinity parameters and identify tumour-reactive T cells that could be further employed for immunotherapy production.
CONCLUSIONS AND FUTURE PERSPECTIVES
Cell immunotherapies based on adoptive cell transfer approaches have developed huge expectations as novel solutions for efficient cancer treatment and eradication. There are however still considerable challenges to be addressed in order to guarantee their widespread administration safely, timely, and at an acceptable cost. Among others, the manufacturing process of cell immunotherapies needs to be updated, incorporating modern cell analysis techniques that allow for a higher automation in the production process and enhancement of the accuracy and reliability of cell biology studies. Optical label-free biosensors are now being profiled as a unique alternative to traditional cell analysis techniques. These biosensor nanotechnologies have demonstrated capabilities for the real-time identification and monitoring of live cell activity without amplification or staining procedures, enabling the evaluation of cell immune responses in a few hours and only using a few cells. Such features could therefore eliminate several cell culture and expansion steps in the manufacturing process, reducing the costs and time delay from months to days. Further investigation might focus on developing new lab-on-a-chip platforms that combine the recent advances in nanophotonics, microfluidics, and bioengineering to eventually deliver more robust, simple, and reliable devices for cell analysis. The upcoming synergy between nanotechnology and biomedical research may be the key for groundbreaking progress and promotion of cell immunotherapies, holding a bright potential for effective and personalised cancer medicine.