Abstract
DNA of human spermatozoa can be subject to various kinds of modifications acquired throughout life. Put simply, two basic types of acquired sperm DNA modifications can be distinguished: genetic and epigenetic. Genetic modifications cause alterations of the DNA sequence and mainly result from the formation of breakpoints leading to sperm DNA fragmentation. Epigenetic modifications include a vast spectrum of events that influence the expression of different genes without altering their DNA sequence. Both the genetic and the epigenetic modifications of sperm DNA can negatively influence embryonic development, cause miscarriages, and be the origin of different health problems for the offspring. As to sperm DNA fragmentation, reliable diagnostic methods are currently available. On the other hand, the detection of potentially harmful epigenetic modifications in spermatozoa is a much more complicated issue. Different treatment options can be chosen to solve problems associated with sperm DNA fragmentation. Some are relatively simple and noninvasive, based on oral treatments with antioxidants and other agents, depending on the underlying cause. In other cases, the recourse to different micromanipulation-assisted in vitro fertilisation techniques is necessary to select spermatozoa with minimal DNA damage to be injected into oocytes. The treatment of cases with epigenetic DNA modifications is still under investigation. Preliminary data suggest that some of the techniques used in cases of extensive DNA fragmentation can also be of help in those of epigenetic modifications; however, further progress will depend on the availability of more reliable diagnostic methods with which it will be possible to evaluate the effects of different therapeutic interventions.
INTRODUCTION
Spermatozoa are vehicles whereby the male genome is transported to the oocyte to participate in syngamy with the female genome and form the resulting embryonic genetic makeup. Compared with the oocyte, sperm DNA needs to be ‘packed’ into the very small sperm nucleus preparative for its delivery to the oocyte. This process requires complex changes in the DNA-associated proteins (sperm chromatin)1 during which sperm DNA is particularly exposed to environmental factors, which can affect its subsequent function in the fertilised oocyte and the resulting embryo.2
In addition to delivering the paternal genetic material to the oocyte, the fertilising spermatozoon is also the source of two developmentally important non-genetic contributions: the factor(s) responsible for the reactivation of the oocyte’s cell cycle, so far arrested at metaphase of the second meiotic division (oocyte activation);3,4 and the centre of microtubule aster formation, required to develop mitotic spindles during the subsequent embryonic cell divisions.5 Dysfunction of any of these factors causes secondary alterations in zygotic and embryonic DNA with possible consequences for further development.
Sperm DNA modifications that affect DNA sequence are called genetic modifications and can be transmitted to the offspring as genetic or ‘hard’ inheritance; however, there are also transmittable acquired sperm DNA modifications that do not affect the DNA sequence but can change the expression pattern of various genes.6,7 These alterations are responsible for what is termed epigenetic or ‘soft’ inheritance. This latter type of inheritance in particular is garnering increasing interest nowadays, and the number of the known epigenetically inherited abnormalities and diseases is growing rapidly.
In this paper, both kinds of acquired sperm DNA changes are addressed, with particular attention given to their causes, pathological consequences, and methods for their prevention and treatment. Due to its clinical focus, a huge body of literature on preclinical research, though important for current knowledge in the field, is omitted.
HOW AND WHERE DO SPERM DNA MODIFICATIONS OCCUR?
Sperm production in the adult human testis begins with the maturation of spermatogonia, the still diploid sperm precursor cells, into primary spermatocytes which enter the first meiotic division, resulting in the formation of secondary spermatozytes. Each secondary spermatocyte subsequently divides, during the second meiotic division, into two haploid round spermatids. The meiotic events leading to the transformation of the diploid spermatocytes into the haploid spermatids are similar to those occurring in oocytes during their maturation. It is only after the completion of meiosis, at the round spermatid stage, when DNA of the future spermatozoon starts undergoing unique changes different from those occurring in oocytes. These changes, necessary to achieve the ‘packaging’ of sperm DNA required for its transportation into the oocyte during fertilisation, make sperm particularly exposed to different kinds of environmental factors which can produce both genetic and epigenetic DNA modifications.2,7
BIOLOGICAL BACKGROUND OF ACQUIRED SPERM DNA MODIFICATIONS
Sperm DNA, similar to oocyte DNA, needs to undergo the process of ‘haploidisation’ whereby the initially diploid precursor cells halve their DNA content in order to be able to restore the normal, diploid chromosomal constitution in the embryo resulting from their fusion at fertilisation. Some harmful sperm DNA modifications can be acquired during the process of haploidisation but most are due to events taking place thereafter, while the haploid round spermatids are being transformed into mature spermatozoa. This is partly because this final phase of sperm development occurs after the release of the sperm precursor cells from Sertoli cells, ‘nursing’ cells that can protect the developing germ cells from adverse external effects and eliminate those germ cells that have been irreversibly damaged.8 Consequently, maturing spermatozoa are increasingly exposed to both genetic and epigenetic modifications during this period.
GENETIC MODIFICATIONS: ALERATION OF SPERM DNA SQUENCE
DNA fragmentation (breakage) is the most commonly acquired sperm genetic modification. Fragmentation is caused by distinct mechanisms and can involve only one of the two complementary strands forming the DNA double helix, thus producing single-strand breaks (SSB), or both of them, resulting in double-strand breaks (DSB). DSB of the DNA molecules occur as a physiological process in prophase of the first meiotic division, when primary spermatocytes deliberately produce DSB to allow DNA recombination between homologous chromosomes.9 These physiological DSB subsequently activate a DNA repair machinery, acting through the protein kinase ataxia-telangiectasia mutated, which repairs the free ends and therefore generates the chiasma.10 Ataxia-telangiectasia mutated is also responsible for preventing the formation of new DSB once the recombination process is accomplished.10 Failures of this DNA repair mechanism at this stage can result in the persistence of DSB which are poorly repaired later during meiosis or after fertilisation.11 In fact, the ability to repair DNA lesions declines dramatically during final stages of spermatogenesis.12 Meiotic interstrand DNA damage that escapes paternal repair can cause chromosomal aberrations in the zygote by maternal misrepair taking place after fertilisation.13
In addition to the physiological, recombination-related DNA breakage, DNA of the developing male germ cell can undergo SSB or DSB through different pathological mechanisms, especially those related to excessive production or insufficient scavenging of reactive oxygen species (ROS).14 Excess ROS can originate both from germ cells themselves and from non-germ cells, and the germ cell chromatin is particularly sensitive to their action during the process of chromatin packaging in spermatids.15
The process of chromatin condensation in late spermatids is accompanied by replacement of DNA-associated histones with protamines (PRM1 and PRM2 in mammals), another type of nuclear protein specifically expressed in haploid male germ cells, whose presence and relative proportion protects sperm DNA from negative influences by environmental factors, and has recently been suggested as a new checkpoint to control sperm chromatin quality.16 Abnormal protamine expression leads to defects in chromatin condensation, occasionally restricted locally.16 The DNA within incompletely condensed areas of sperm nuclear chromatin, which can be visualised as intranuclear vacuoles by electron microscopy (Figure 1), appears to be the most vulnerable to oxidative damage, especially after late spermatids separate from Sertoli cells (spermiation) leading to the loss of these nursing cells’ protective action.8
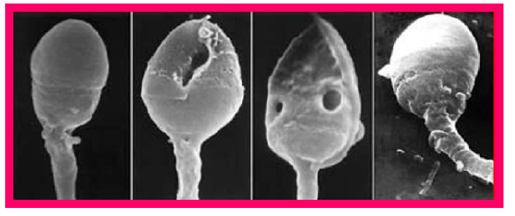
Figure 1: Scanning electron microscopy (SEM) image of human heads. The two middle images show the presence of intranuclear vacuoles.
According to several recent reviews,17-19 including one meta-analysis,20 sperm DNA lesions have been shown to have negative consequences for embryogenesis after in vitro fertilisation, reflected by impaired morphological quality of Day 3 embryos,17,18 increased incidence of embryonic cell apoptosis,19 and decreased blastocyst formation, implantation, and pregnancy rates.17,18,20
There are a number of aetiological factors that can contribute to the pathogenesis of sperm DNA fragmentation. Different studies have suggested an association of sperm DNA breakage with age;21 lifestyle habits such as dietary preferences,22 smoking,23-26 chronic alcoholism,27 or recreational drug addiction;28,29 environmental factors;30 various diseases such as varicocele; 31,32 infections;33-35 spinal cord injury;36,37 diabetes38 and obesity;39 and drug therapies, including cancer chemotherapy40,41 and antidepressants.42,43 Most of these factors act via an excess of ROS, as first demonstrated in 1987.44
Even though small amounts of ROS are normally produced by spermatozoa themselves and are essential for the fertilisation process, their excessive production by abnormal spermatozoa or by leukocytes in response to inflammation and infection, or their insufficient scavenging by antioxidant agents naturally present in human semen, result in ROS imbalance which leads almost invariably to increased sperm DNA damage.45 On the other hand, some pathological conditions can also cause excessive sperm DNA fragmentation independently of ROS.46
EPIGENETIC MODIFICATIONS: ALTERATION OF SPERM DNA FUNCTION
Sperm DNA function can be altered, while keeping its sequence intact, by the action of complex mechanisms referred to as epigenetic modifications.15 The most widely investigated epigenetic modifications include DNA methylation taking place at the 5’ position of cytosine in CpG dinucleotides and histone acetylation.6,47 However, a number of other epigenetic factors have been described and are critical in regulating gene expression, including chromatin structure and the expression of small non-coding RNA, such as microRNA, small interfering RNA, and Piwi-interacting RNA.7,48
Epigenetic information carried in sperm in the form of histone modifications, transcriptional factors, and chromatin three-dimensional architecture are other players in this complex game.49 It is now clear that some of these epigenetic signatures persist after fertilisation, through different mechanisms, and influence future zygote, embryonic, fetal, and offspring development.50-54
These considerations are particularly important in the current era of assisted reproductive technologies (ART). Nowadays, ART can facilitate the transmission of these kind of factors by enabling fertilisation with abnormal spermatozoa. In the near future, however, newly emerging techniques will be likely to reduce the risk of epigenetic disease transmission by quality control of spermatozoa selection for fertilisation via detection of epigenetic biomarkers.55 Out of the 6,871 sperm proteins identified so far, 560 have been found to be involved in modulating gene expression by transcription regulation, DNA methylation, histone post-translational modification, and non-coding RNA biogenesis.56 It is in this group of proteins in which suitable candidates for such epigenetic biomarkes can be searched for, however direct analysis of DNA and RNA isolated from spermatozoa and seminal plasma is also a promising approach.57
The demonstration of the existence of epigenetic inheritance explains a number of clinical observations which would be hardly attributable to genetic inheritance only. For instance, in spite of an irrefutable evidence for an elevated germline mutation rate in patients directly exposed to ionising radiation, the results of numerous studies suggest that the incidence of the observed effects on genomic instability in the offspring is too high to be explained merely by radiation-induced mutations, which occur at a substantially lower rate.58 Epigenetic inheritance through gametes can also explain both previously published and more recent data on transgenerational transmission of obesity,59-61 diabetes,62 and some types of cancer.63,64 In addition to these ‘metabolic’ issues, recent research data show that early life stress in humans (e.g., maltreatment, violence exposure, loss of a loved one) and in rodents (e.g., disrupted attachment or nesting, electric shock, restraint, predator odour), a condition associated with increased risk of developmental psychopathology, can also be transmisible to subsequent generations via DNA methylation marks.65
CLINICAL CONSEQUENCES OF SPERM DNA MODIFICATIONS
Both genetic and epigenetic acquired sperm DNA modifications can have serious consequences for the survival and health status of embryos, fetuses, and the offspring.
Genetic Modifications
Alterations of the sperm DNA sequence can disturb different, developmentally important genes. Some of these alterations can be repaired by mechanisms acting in the germline or in the zygote after fertilisation.66 The repair capacity of the testicular germinal epithelium appears to be related to the correct function of Sertoli cells. In fact, Sertoli cells not only participate in the germ cell DNA repair but also remove, by phagocytosis, those germ cells that cannot be repaired.67 If Sertoli cell function is disturbed, more germ cells with damaged DNA can escape this output control, complete spermatogenesis, and eventually be released in the ejaculate.67 In vitro depletion of testosterone in culture media was shown to induce Sertoli cell apoptosis in human seminiferous tubules obtained from men with obstructive azoospermia and normal spermatogenesis.68,69 This observation can explain the mechanism of action of some aetiological factors causing sperm DNA damage mentioned above, many of which are associated with decreased testicular testosterone production.70
Those DNA-damaged spermatozoa that escape the intratesticular quality-control mechanisms can be found in the ejaculate. In many cases sperm DNA damage is associated with other sperm functional disturbances which decrease their capacity to fertilise the oocyte by proper means. However, fertilisation with such spermatozoa is more likely when fertilisation is carried out by intracytopasmic sperm injection (ICSI). Sperm DNA damage can still be repaired after fertilisation through different mechanisms acting in the zygote.66 It was noted that embryos resulting from fertilisation with spermatozoa from men with the same degree of DNA damage are more likely to develop to term when injected into the oocytes of very young women, such as oocyte donors whose maximum age is 25 years at the author’s clinic, compared with older women.8 Consequently, the capacity of the zygotic DNA repair appears to depend on the age-related oocyte quality.
If sperm DNA damage is not repaired, fertilisation usually takes place and the embryonic development goes on normally during the first 3 days after fertilisation.71 The absence of apparent effects of unrepaired DNA damage on the early embro is likely to be related to the relatively late onset of paternal gene expression which occurs between the 4-cell and 8-cell stage in human embryos.72-74 Moreover, oocyte-derived transcripts continue participating in the control of embryo development even during later stages of preimplantation development, including blastocyst formation.75 On the other hand, sperm DNA fragmentation is associated with embryo implantation failure and miscarriage, referred to as the late paternal effect.76 These problems occur at stages when the embryo becomes exclusively dependent on its own gene expression, and the timing of the onset of the late paternal effect clearly depends on the type and number of genes affected by sperm DNA fragmentation. Embryo aneuploidy, resulting from mismatch zygotic repair of sperm DNA strand breaks,13 can also contribute to the late paternal effect. A recent study has suggested that DSB of sperm DNA are more likely to cause a delay in embryo development and to cause implantation failure as compared with SSB.77
Epigenetic Modifications
The first studies into transgenerational epigenetic inheritance were focussed on imprinted genes.78-81 Imprinted genes are those that have to be expressed or silenced in a parent-of origin manner in order to allow normal dvelopment.82 Several human diseases, such as Beckwith–Wiedemann syndrome, Silver–Russel syndrome, Prader Willy syndrome, and Angelman syndrome are caused by imprinted gene disruption leading to their abnormal expression.82 Of these, an increased incidence of Beckwith–Wiedemann syndrome and Silver-Russel syndrome appears to be associated with assisted reproduction.83,84
A systematic review and meta-analysis of the literature has revealed a higher risk of congenital disorders caused by disruption of imprinted genes in children conceived through ART compared with those conceived naturally.84 There is evidence from human studies that a number of assisted reproduction procedures, including superovulation, gamete micromanipulation, in vitro maturation of oocytes, and embryo culture can cause epigenetic disruption which is likely to involve both imprinted and non-imprinted genes.79 The epigenetic risks associated with ART are thus not limited to abnormalities of imprinted genes but can concern a wide range of non-imprinted genes, leading to their abnormal expression and ensuing pathologies.85
In contrast with genetic modifications, epigenetic modifications of sperm DNA usually affect embryo development from its very beginning and are referred to as the early paternal effect.71,76 In many cases, the early paternal effect can be detected as early as the one-cell zygote stage, based on the analysis of the assembly of nucleolar precursor bodies (NPB) in the male and the female pronucleus.86 Ultrastructural and autoradiographic studies on NPB assembly in human zygote pronuclei87,88 detected the presence of new RNA synthesis in the chromatin regions adjacent to the NPB, and this early zygotic RNA synthesis was required for the NPB formation to go ahead.89 Because, as mentioned in the previous section, the expression of the human embryonic genome starts much later during preimplantation development,71-74 it is reasonable to suppose that the RNA synthesised in human zygotes might correspond to non-coding RNA species, such as microRNA, small interfering RNA, and Piwi-interacting RNA. These non-coding RNA are known to be involved in epigenetic events modifying gene activity.7 This might explain the observed relationship between abnormalities of NPB in human zygotes and subsequent developmental fate of the respective embryos.86,90 Moreover, the regulatory and amplifying activities of non-coding RNA acting during the early stages of embryonic development appear to be conditioned by factors present in the female genital tract.91 Clearly, the possible repair mechanisms involving these factors during early zygote and preimplantation embryo development are precluded when the early embryos develop under in vitro culture conditions.
Previous findings have shown that the assembly of NPB coincides with the assembly of microtubule organising centres in human zygotes,92,93 a process whose perturbations can cause abnormal chromosome segregations during embryo cleavage divisions, leading to aneuploidy. This can explain the observation that abnormal patterns of NPB assembly can also predict blastocyst transfer outcome94 and chromosome constitution.95 As previously mentioned, epigenetic mechanisms also appear to be responsible for transgenerational transmission of obesity,60,61 diabetes,62 and some types of cancer;63,64 however, the mechanism of these phenomena remains to be elucidated.
PREVENTION OF HARMFUL SPERM DNA MODIFICATIONS
Both the genetic and the epigenetic sperm DNA modifications responsible for human disease have some common features. A recent study using cattle as the model showed that increased exposure of spermatozoa to oxidative stress causes changes in the sperm chromatin that are associated with both DNA fragmentation and aberrant DNA methylation affecting epigenetic reprogramming in early embryonic development.96 Studies conducted with human spermatozoa showed that oxidative sperm DNA damage does not affect all of the sperm DNA in the same way. DNA located in compacted regions of the sperm nucleus, in which the process of sperm chromatin condensation has been completed sucessfully, is less exposed to oxidative damage compared with that located in incompletely compacted chromatin regions. This applies both to DNA fragmentation97 and abnormal DNA methylation.98 As oxidative stress affects sperm DNA both directly and indirectly, through impairing sperm chromatin condensation (Figure 1), eliminating the sources of excess ROS is the main preventive measure (Table 1).
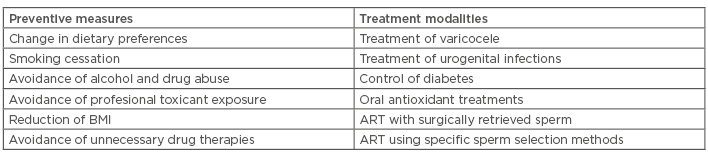
Table 1: Summary of preventive measures and treatment modalities which can improve fertility in cases of acquired genetic and epigenetic sperm DNA modifications.
ART: assisted reproductive technologies; BMI: body mass index.
Excessive ROS can be of both endogenous (sperm-derived) and exogenous in origin.
The aetiological factors leading to excessive ROS production include unhealthy dietary preferences,17 smoking,18-21 chronic alcoholism,22 recreational drug use,23,24 varicocele,26,27 infections,28-30 spinal cord injury,31,32 diabetes,33 obesity,34 and drug therapies including cancer chemotherapy35,36 and antidepressants.37,38 If any of these conditions are present in an infertile patient showing abnormally high levels of sperm DNA fragmentation, the first measure to be taken is to try and treat the underlying cause.99 If this is not possible, or the measures taken do not give an expected result, the negative impact of ROS can be reduced by the use of scavenging agents, mainly antioxidants such as vitamin C, vitamin E, L-carnitine, coenzyme Q10, zinc, selenium, vitamin B9, vitamin B12, glutathione, docosahexaenoic acid, and folic acid.100 Exogenous melatonin supplementation also prevents oxidative stress-evoked DNA damage in human spermatozoa.101 If the patient shows abnormal levels of reproductive hormones, especially testosterone, follicle stimulating hormone, or luteinising hormone, a condition that can disturb the function of Sertoli cells may manifest, leading to an insufficiency of intratesticular germ cell DNA repair mechanisms. Hormonal replacement therapy can also be considered. In cases of infection, revealed by the detection of germs or excess leukocytes in the ejaculate, the use of specific antibiotics, sometimes accompanied by nonsteroidal anti-inflammatory drugs, can be of help. Appropriate personalised treatment of diabetes can improve the diabetes-associated sperm DNA modifications. If the use of none of these preventive measures improves the degree of sperm DNA alteration, or if none of the above potential aetiological factors can be detected, more radical treatment options (see below) can be taken into consideration. However, it is evident that the physician cannot efficiently control all of the factors related to excessive sperm DNA damage, and in some conditions, such as chemotherapy or antidepressant/antipsychotic medications, the physician’s decision should be guided by the ‘primum non nocere’ (first, do not harm) principle, in agreement with the Hippocratic Oath. The possible risks and benefits of the interruption of the treatment in course have to be weighed. If the interruption of sperm DNA-damaging medication is not advisable, the recourse to ART, employing specific in vitro treatment techniques to improve outcomes in cases of excess DNA damage, can be envisaged.
TREATEMENT POSSIBILITIES
In some cases in which the appropriate treatment of the pathologies underlying the extensive sperm DNA damage (Table 1) does not lead to a significant improvement or in which no such aetiological factors can be identified, the treatment with high doses of oral antioxidants during several months can also be of help.102 The resulting improvement can be sufficient to allow natural fertilisation but the recourse to ART is often required. The decision of whether or not assisted reproduction should be used in different clinical scenarios is sometimes difficult and depends on a number of associated factors concerning both the male and the female partner of the infertile couple. Recently, a guideline for the choice of the optimal assisted reproduction techniques according to differerent criteria has been suggested, including the basic sperm parameters, the duration of infertility, the number and type of previous unsuccessful assisted reproduction attempts, and the female age.103 The techniques available include conventional ICSI, intracytoplasmic morphologically selected sperm injection (IMSI),104,105 ‘physiologic ICSI’,106 or a combination.103 In case of failure of these noninvasive techniques, based on laboratory workup, ICSI with surgically retrieved testicular spermatozoa can solve the problem.107
As to the treatment of harmful epigenetic sperm DNA modifications, they are often associated with sperm chromatin abnormalities, and IMSI appears to be the method of choice to select spermatozoa with normal epigenetic profile to be used for fertilisation.98 Hence, the use of IMSI appears to be particularly interesting for the prevention of both genetic and epigenetic acquired sperm DNA alterations. In fact, IMSI allows the detection of regions with defective sperm chromatin condensation, appearing as intranuclear vacuoles (Figure 2) with a precision similar to that achieved by electron microscopy (Figure 1). The processing of specimens for electron microscopy is incompatible with cell survival, whereas IMSI can be used to select living, vacuole-free spermatozoa to be injected into oocytes, with a resolving power highly superior to that of the conventional ICSI procedure (Figure 3). All of the above techniques have been reported to be successsful in some cases of excess sperm DNA damage.
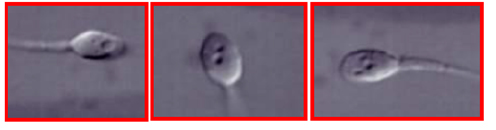
Figure 2: Sperm intranuclear vacuoles observed in living human spermatozoa at the magnification of x6,500, as used in intracytoplasmic morphologically-selected sperm injection (IMSI).
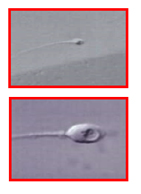
Figure 3: A human spermatozoon observed at the conventional magnification used in intracytoplasmic sperm injection (ICSI) (upper panel), and at high magnification used in intracytoplasmic morphologically-selected sperm injection (IMSI) (lower pane). The large intranuclear vacuole seen in the IMSI system is not detectable using the conventional ICSI magnification.
However, the usefulness of each of them, in the context of different clinical scenarios, still remains to be confirmed by larger clinical studies.
FUTURE PERSPECTIVES
In spite of the wide range of diagnostic and therapeutic methods currently available to be used in cases of sperm DNA fragmentation, there are relatively few techniques for the management of epigenetic alterations of sperm DNA. As several waves of microRNA and tRNA fragments, molecules known to be involved in epigenetic modifications of DNA,7 have been shipped to sperm during post-testicular maturation in the epididymis,108 it remains to be determined whether the epigenetic status of human ejaculated spermatozoa differs from that of testicular spermatozoa. If RNA of epididymal origin responsible for epigenetic damage can be identified, they can be used for the development of diagnostic tests which might point out the cases of epigenetic sperm DNA damage in which the use of testicular spermatozoa would be of help.
Other, more sophisticated technologies, such as injecting specific microRNA molecules capable of repairing specific epigenetic defects into the early zygote,108 or induction of DNA methylation of the genes of interest by a Dnmt3-type de novo DNA methyltransferase targeted to the corresponding sperm DNA sequence by a nuclease-inactivated CRISPR variant (dCas9),109 may also be explored.